
Lipid Nanoparticles
Introduction to Lipid Nanoparticles
Lipid nanoparticles (LNPs) represent a cutting-edge and versatile technology in the field of drug delivery and gene therapy. LNPs exploit the unique properties of lipids including their biocompatibility and ability to interact with cell membranes. These features enable them to encapsulate and transport a wide range of bioactive molecules including small-molecule drugs, nucleic acids and proteins. Thus, LNPs hold great promises for addressing the limitations of conventional drug delivery methods and have the potential to revolutionise the treatment of various diseases (Torchilin, 2014). Here we provide a short summary of the structure, different types, methods of preparation, the encapsulation process, and the applications of LNPs.
​
​
LNPs are a lipid based core surrounded by a lipid layer forming a spherical or slightly ellipsoidal shape (Torchilin, 2014). They are made up of four distinct parts:
​
- Ionizable lipids which allow the LNPs to adjust to the acidic environment inside the endosomes and initiate drug release. Under acidic conditions, these lipids become positively charged, promoting endosomal escape. This means that the ionisation of these lipids increases the destabilisation of endosomal membranes, allowing encapsulated drugs to be released in the cytoplasm (Zuhorn & Hoekstra, 2002; Jung et al., 2022; Hald Albertsen et al., 2022).
​
- Phospholipids, termed 'helper lipids', which provide structural stability that aid in cytosolic entry and intracellular uptake (Cheng & Lee, 2016; Jung et al., 2022; Hald Albertsen et al., 2022).
​
- Cholesterol which promotes the formation of LNPs, improves their stability by controlling membrane rigidity and integrity and improves gene transfection and biodistribution (Cullis & Hope, 2017; Jung et al., 2022; Hald Albertsen et al., 2022).
​
- Poly(ethylene glycol) (PEG) lipids which have a hydrophilic head and a hydrophobic tail, that increase LNP stability, reduce aggregation by acting as a steric barrier, shield the surface of the LNPs and control the stable particle size (Holland et al., 1996; Bao et al., 2013; Jung et al., 2022; Hald Albertsen et al., 2022).
What are LNPs?
The efficacy of LNPs is strongly influenced by the ratios of ionizable lipids, helper lipids, cholesterol, and PEG lipids, which must be optimised for particular applications and delivery methods. The action of these nanoparticles inside the body is also influenced by the type of lipid, nanoparticle size, and surface charge (Let’s talk about lipid nanoparticles, 2021).
​
​
​
​
Figure 1: LNP structure composed of PEG-lipids on the surface, cationic ionizable lipids, cholesterol and helper lipids, by Kularatne et al., (2022).
​
Lipid nanoparticles have a number of benefits (Ghasemiyeh & Mohammadi-Samani, 2018; Tenchov et al., 2021). These include:
1. Large-scale production
2. Biodegradable nature of the materials
3. Low toxicity potential - lipids used in these nanoparticles are biocompatible and completely tolerated by the body like triglycerides, fatty acids, steroids, and waxes.
4. Possibility of controlled and modified drug release - they have the ability to control the location and timing of drug delivery in the body.
5. Drug solubility enhancement and the possibility of both hydrophilic and lipophilic drug incorporation.
6. Targeted delivery-with their ability to encapsulate and deliver therapeutics to specific locations within the body and to release their contents at a desired time, LNPs provide a valuable platform for treatment of a variety of diseases.
7. Versatility - they are able to deliver a variety of therapeutic agents such that the application of LNPs has also been extended to other fields, such as medical imaging, cosmetics, nutrition, agriculture, and other innovative areas such as nanoreactors.
Despite their immense potential, LNPs also present challenges and limitations such as:
-
Low oral bioavailability
-
Problems with absorbance of the particles.
​
Ongoing research is dedicated to optimising their design, formulation and safety to harness their full potential while minimising risks (Mohammadi-Samani & Ghasemiyeh, 2018).
​
​
Depending on the type of LNP, the lipid core may be solid (solid lipid nanoparticles) or a combination of solid and liquid lipids (nanostructured lipid carriers) (Torchilin, 2014).
​
​
​
​
​
​
​
​
​
​
Figure 2: Three different types of LNPs: a) liposomes, b) SLNs and c) NLCs, by Xu et al., (2022).
​
Liposomes, an early form of LNPs, are a very flexible nanocarrier platform as they are able to transport both hydrophobic and hydrophilic materials, particularly proteins, small molecules and nucleic acids (Bangham et al., 1965; Bangham et al., 1967; Tenchov et al., 2021).
​
Liposomes are spherical particles made up of phospholipid molecules within an aqueous core that combine to form contiguous membrane bilayers capable of entrapping solute. LNPs, on the other hand, may or may not properly encapsulate a solute within the aqueous compartment. Unlike liposomes, which have a lipid bilayer membrane with diameters ranging from tens of nanometers to micrometres, not all LNPs have a bilayer that could classify them as vesicles or liposomes (Bangham et al., 1965; Kraft et al., 2014; Naseri et al., 2015).
​
With a number of approved pharmaceutical formulations, liposomes were the first nanomedicine delivery medium to successfully transition from concept to clinical application (Gregoriadis et al., 1971; Gregoriadis a, 1976a; Gregoriadis b, 1976b; Tenchov et al., 2021).
​
The following table shows the advantages and disadvantages of liposomes (Naseri et al., 2015):
​
​
​
​
​
​
​
​
​
​
​
​
​
Table 1: Advantages and Disadvantages of Liposomes
​
​
​
Solid Lipid Nanoparticles (SLNs), ranging in size from 40 to 1000 nm, emerged as an alternative to polymeric nanoparticles, emulsions, and liposomes. They consist of solid fat that makes up between 0.1 to 30 (% w/w) dispersed in water which comes from fatty acids, steroids, waxes, monoglycerides, diglycerides, and triglycerides. Surfactants are utilised to improve stability of the nanoparticles. The proper combination of lipids and surfactants impacts the particle size and drug loading capabilities. SLNs may be analysed by Transmission electron microscopy and Scanning Electron Microscopy (Lucks & Müller, 1991; Pardeike et al., 2009; Naseri et al., 2015).
The table below presents the advantages and disadvantages of SLNs (Naseri et al. 2015):
​
​
​
​
​
​
​
​
​
​
​
Table 2: A table showing the advantages and disadvantages of SLNs.
​
​
​
Nanostructured lipid carriers (NLCs), ranging in size from 50-500 nm, are modified generations of SLNs which contain both solid (fat) and liquid (oil) lipids at room temperature (Müller et al. a, 2002a; Müller et al. b, 2002b; Naseri et al., 2015; Khan et al., 2023). There are three forms of NLCs: the formless type (non-crystalline matrix), the multiple type (higher drug solubility in liquid lipid than solid lipid), and the imperfect type (mixing solid and liquid fats) (Müller et al. a, 2002a; Müller et al. b, 2002b; Naseri et al., 2015).
​
The table below presents the advantages and disadvantages of LNCs compared to SLNs (Naseri et al., 2015; Khan et al., 2023; Jaiswal et al., 2016):
​
​
​
​
​
​
​
​
Table 3: A table showing the advantages and disadvantages of NLCs compared to SLNs.
Types of Lipid Nanoparticles
Liposomes:
Advantages | Disadvantages |
---|---|
Low toxicity | Difficulties in interaction between cells and intermembrane transfer |
Non-immunogenic
| Quick clearance by the reticuloendothelial system (RES) |
Easily Degradable | Poor stability |
Flexible nature | Poor encapsulation efficiency |
Biocompatible | Short shelf life |
Protection of drugs from enzymatic breakdown |
Solid Lipid Nanoparticles
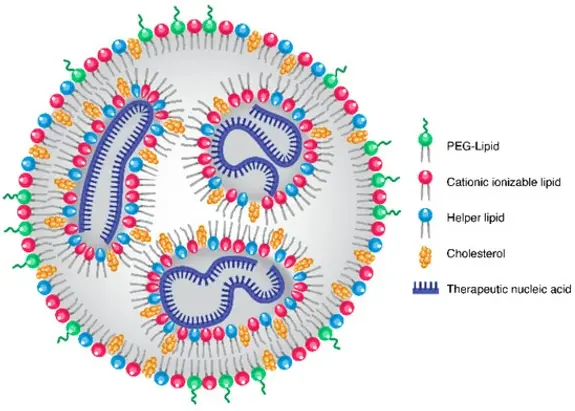

Advantages | Disadvantages |
---|---|
Inexpensive | Due to crystalline form of the solid lipid:
|
Simple preparation | - Difficulties in lipid particle development |
Large-scale production | - Tendency for gelation |
Biodegradability | - Low integration rate |
Chemical adaptability |
Nanostructured Lipid Carriers
Advantages compared to SLNs | Disadvantages |
---|---|
Reduced drug expulsion by preventing lipid crystallisation during storage | Surfactants irritation |
Higher loading capacity for drugs due to irregular crystal structure | Potential cytotoxic effects |
Improved stability
|
Lipid Nanoparticle Preparation
Multiple methods have been found to result in the production of lipid nanoparticles. These include high energy methods such as high pressure homogenization (hot and cold) and ultrasonic solvent emulsification, low energy methods such as microemulsion formation, and organic-solvent based methods such as solvent emulsification/evaporation (Dhiman et al., 2021).
​
​
High pressure homogenization is the most common and effective method used to prepare lipid nanoparticles. The homogenization can either be hot or cold and is a high energy technique that uses a high pressure to press a liquid through a gap of a few microns (Dhiman et al., 2021; Ghasemiyeh & Mohammadi-Samani, 2018; Mehnert & Mäder, 2001)
-
Hot high pressure homogenization
This method is typically used for heat stable materials where the drug is first introduced into the lipid which is then heated to a temperature that does not exceed 90 °C. A pre-emulsion is then formed by dispersing the hot lipid phase in an aqueous surfactant solution with the same temperature. This solution is then allowed to undergo cycles of high pressure for homogenization. The resultant oil in water microemulsion is then cooled at room temperature and allowed to solidify into SLNs or NLCs. This method has the benefit of allowing the inclusion of insoluble and lipophilic drugs, however, the incorporated drug can be degraded due to the high temperatures used in this technique (Dhiman et al., 2021; Ghasemiyeh & Mohammadi-Samani, 2018; Schwarz et al., 1994).
​
-
Cold high pressure homogenization
This technique is mainly used for thermo-labile drugs. The drug is first introduced into a melted lipid phase and is cooled promptly in dry ice or liquid nitrogen to solidify. This is then crushed to obtain lipid microparticles that are added in a cool aqueous phase consisting of surfactants to result in a pre-suspension. The pre-suspension is then subjected to cycles of high pressure at room temperature in order to be homogenised. This technique can reduce the drawbacks of the hot homogenization method as it eliminates the use of high temperatures which can degrade the incorporated drugs (Dhiman et al., 2021; Ghasemiyeh & Mohammadi-Samani, 2018; Mehnert & Mäder, 2001).
​
​
Solvent emulsification/evaporation is the most commonly used method for the preparation of NLCs. In this method, the lipid phase and the drug are dissolved in a non-polar organic solvent such as chloroform. The mixture is then added to an aqueous phase containing a surfactant under high-speed and constant stirring at 70-80 °C till the organic phase evaporates completely. The obtained nanoemulsion is then cooled to solidify the lipid nanoparticles. The major limitation of this method is the use of an organic solvent which may limit lipid solubility in the organic phase and interact with drug molecules (Dhiman et al., 2021; Ghasemiyeh & Mohammadi-Samani, 2018; Sjöström & Bergenståhl, 1992).
​
​
Microemulsions are composed of aqueous and oil phases. These biphasic systems are formed by stirring a mixture of emulsifier, co-emulsifier, lipid, and water at 65–70°C. The obtained hot microemulsion is placed in cold water kept at 2–3°C under constant stirring to obtain a lipid suspension. This suspension is then washed and filtered, obtaining the final SLNs. The major limitation of this method is a low production yield (Dhiman et al., 2021; Gasco, 1997; Ghasemiyeh & Mohammadi-Samani, 2018).
​
​
In the ultrasonic solvent emulsification method, an organic solvent such as dichloromethane is used to dissolve the lipid phase and heat it to a temperature no higher than 50 °C. An aqueous phase, containing emulsifiers and surfactants, is brought up to temperature and added to the partially evaporated organic phase. The resulting emulsion is then subjected to sonication for a set time. Lastly, it is placed to cool in an ice bath in order for the lipid nanoparticles to solidify (Eldem et al., 1991; Ghasemiyeh & Mohammadi-Samani, 2018).
​
The following table entails a summary of the advantages, and disadvantages of the methods mentioned (Dhiman et al., 2021; Garud et al., 2012; Mehnert & Mäder, 2001):
​
​
​
​
​
​
​
​
​
​
​
​
​
​
​
​
Table 4: A table showing the advantages and disadvantages of the methods of preparation of LNPs.
High pressure homogenization technique
Solvent emulsification/evaporation technique
Microemulsion formation technique
Ultrasonic solvent emulsification technique
Method | Advantages | Disadvantages |
---|---|---|
Ultrasonic solvent emulsification |
|
|
Microemulsion formation |
|
|
Solvent emulsification/ evaporation | No high temperatures are used | Use of organic solvents which may limit lipid solubility in the organic phase and interact with drug molecules |
Cold high pressure homogenization |
| Broader size of the resulting nanoparticles |
Hot high pressure homogenization | Small particles obtained |
|
LNPs Encapsulation Process
To utilise the great properties of LNPs, the initial step involves encapsulating, or placing materials inside, the LNPs. Depending on the ultimate goal, LNPs can effectively deliver either genetic material or drugs.
​
​
LNPs are gaining popularity as nucleic acid delivery systems due to their ability to intracellularly deliver while shielding nucleic acid molecules like DNA, RNA or small interference RNA (siRNA) from the severely damaging nuclease environment in vivo (Mukai et al., 2022; Brader et al., 2021). The delivery process involves a number of steps, starting with selecting the right lipid for constructing the LNPs. The delivery process is summarised below:
​
1. Lipid selection and formation
The procedure begins with the selection of appropriate lipids or lipid combinations for the formation of lipid nanoparticles. These lipids are intended to preserve nucleic acids while also facilitating their transport into target cells (Cullis & Hope, 2017).
​
In order to design non-viral gene delivery vehicles, efficient packing of the genetic molecules inside the cells must be ensured, with using either lipid or surfactant assemblies or polymers. The large negative charge on the nucleic acids can thus be masked, allowing transport across the cellular membrane to occur. To control the charge and the liquid crystalline phase of the lipid-based systems, neutral helper lipids can be used. Cuboplexes that are cubosomes that deliver nucleic acids to cells can be formed with mRNA, for example, by including lipids that self-assemble into bicontinuous gyroid - a triply repeated minimal surface - liquid crystalline phases, such as glycerol monoleate (Cárdenas et al., 2023; Shoen, 1970; Oliveira et al., 2022; Kim & Leal, 2015).
​
It is also critical to dissociate or decompact the nucleic acids once the gene delivery vehicle containing it enters the target cell. Simultaneously, it is essential to reduce the toxicity of the permanently charged cationic molecules used as compacting agents. Several new lipids and surfactants have been designed including disulfide cationic lipids, which break the disulfide bond at low pH. This causes the cleavage of the cationic group of the lipid. Cationic ionizable lipids with the positive charge at low pH can also be used, enabling the collapse of lipid-based systems (Cárdenas et al., 2023).
​
The physiological characteristics of the produced nanoparticles are carefully characterised, including size, surface charge, and encapsulation effectiveness. These characteristics are critical in defining the delivery system's efficacy and safety (Semple et al., 2010; Yin et al., 2014).
​
2. Complexation of nucleic acids
Nucleic acids, such as DNA or RNA, are complexed with the lipid formulation of choice. Because of the positive charge at low pH of ionizable lipids, LNPs can interact with anionic nucleic acids. This guarantees that the nucleic acids are contained within the lipid nanoparticles, resulting in stable complexes that can be successfully administered (Semple et al.,2010; Hald Albertsen et al., 2022).
​
3. Cellular uptake and endosomal escape
Target cells internalise the lipid nanoparticles via endocytosis. By interacting with cell membranes, the ionizable lipid component increases cellular uptake (Hald Albertsen et al., 2022).
​
In order to allow the nucleic acids to reach the cytoplasm and accomplished endosomal escape for nucleic acid release different approaches can be applied, including creation of cationic lipids and use of pH-sensitive lipids that are neutral at physiological pH (Sun & Lu, 2023).
​
4. In vivo administration and therapeutic efficacy
The optimised lipid nanoparticles are delivered in vivo to assess their efficiency in delivering nucleic acids to specific tissues or cells. This stage entails evaluating the therapeutic effects and ensuring that the nucleic acids given perform as planned (Hajj & Whitehead 2017).
​
​
​
​
​
​
​
​
​
​
​
​
​
​
​
​
Figure 3. Process of encapsulation and delivery of mRNA with LNPs: (1) contact between the delivery system and cell membrane, (2) endocytosis and (3) endosomal escape with the release of mRNA, by Aldosari & Alfagih (2021).
​
​
​
Lipid nanoparticles have emerged as a promising drug delivery system, providing a versatile and efficient way to improve the therapeutic efficacy of a variety of drugs. LNPs offer a stable and biodegradable platform for encapsulating a wide variety of drugs, protecting them from degradation and allowing for controlled release (Bangham et al., 1965; Bangham et al., 1967; Müller et al., 2000; Xu et al. 2021; Leong & Ge, 2022). LNPs have been extensively researched for their use in drug delivery, including small molecules and proteins, demonstrating their potential across a wide range of therapeutic areas (Puri et al., 2009; Xu et al., 2021; Leong & Ge, 2022).
​
Lipid nanoparticles as a drug delivery system entails several key steps:
1. Formation of LNPs
To begin, LNPs are created using techniques including high-pressure homogenization, microemulsion, or solvent evaporation. The choice of appropriate lipids is critical in determining LNP stability and biocompatibility (Mehnert & Mäder, 2001; Müller et al., 2000; Xu et al., 2021).
2. Encapsulation of drugs
Drugs are encapsulated within LNPs during the formation, protecting them from degradation and increasing their bioavailability. The physicochemical properties of both the drug and the chosen lipid components influence the encapsulation process (Müller et al., 2000; Scioli Montoto et al., 2020; Xu et al., 2021).
​
3. Intracellular Drug Release
LNPs facilitate the intracellular release of encapsulated drugs once they reach the target cells. Endocytosis and subsequent release from endosomal compartments are two mechanisms that can occur. LNPs with triggered release mechanisms, such as pH-sensitive components or temperature-responsive lipids, can be designed to allow for controlled drug release within the intracellular environment (Torchillin, 2010; Akita et al., 2023).
​
4. In vivo Administration
Once loaded with drugs, LNPs are designed for in vivo administration, with particle size, surface charge, and targeting ligands influencing biodistribution and pharmacokinetics (Allen & Cullis, 2013; Scioli Montoto et al., 2020).
​
​
The applications and versatility of LNP technology are underscored by various studies. These range from chemotherapeutic drug delivery systems, RNA sequence analysis for personalised medicine, refined messenger RNA (mRNA) and small interfering RNA (siRNA) delivery to the treatment of neurological disorders.
LNPs are increasingly being explored for the targeted delivery of chemotherapeutic agents where they can enhance the solubility and bioavailability of the drug and reduce off-target effects. This is particularly advantageous in the treatment of cancers and other diseases where traditional chemotherapy has been limited by systemic toxicity.
The use of LNPs extends beyond drug delivery to diagnostics and imaging. They can be loaded with contrast agents for medical imaging making them a valuable tool for non-invasive disease detection and monitoring (Cullis & Hope, 2017). An innovative use of delivering nucleic acids to cells is the delivery of antibody-encoding mRNA to targeted cells, which results in the expression and production of the specified antibodies, mounting an immune response against the antigen (Han et al., 2023). For example, LNPs are used to deliver mRNA encoding Tumour-associated antigens (TAAs) to malignant cells. This has been performed on T-cells, transfecting them with mRNA which encodes CD19-CAR which results in the activation of cell mediated immunity processes against malignant cells. This treatment holds tremendous promise for personalised and effective cancer therapy. However, this form of treatment is limited by the short duration in which the genes are expressed. The addition of immunologic adjuvants (an ingredient used in some vaccines that helps create a stronger immune response) into the LNPs has shown to be successful in mouse studies, leading to reduced tumour growth and increased chances of survival (Han et al., 2023).
One of the most significant applications of LNPs is in the delivery of RNA-based therapeutics, including mRNA and siRNA.The extensive applications of LNP-mRNA technology have become evident with the rapid development and authorization of mRNA vaccines such as the COVID-19 vaccines which utilise LNPs for efficient mRNA delivery. LNPs protect the fragile RNA molecules from degradation and facilitate their entry into target cells enabling the translation of genetic instructions into protein synthesis (Pardi et al., 2018).
As mentioned, SLNs have emerged as promising tools for drug delivery in the realm of CNS-related disorders. Numerous research studies have explored their potential in delivering medication to patients with neurological conditions. In a study by Zhan M., SLNs were employed to encapsulate Levodopa, a drug targeting the dopaminergic receptors crucial in Parkinson's disease. This approach aimed to enhance its bioavailability and, consequently, its therapeutic effectiveness (Zhan et al, 2010). Similarly, SLNs have been utilised to facilitate the delivery of other Parkinson's drugs across the blood-brain barrier (BBB). Beyond Parkinson's, SLNs have been investigated for their ability to transport drug candidates across the BBB to target Huntington's Disease. These lipid nanoparticles are also showing promise in drug delivery for epilepsy, ischemic stroke, and various neurodegenerative diseases, with encouraging results observed in in vivo rat models. The use of SLNs offers exciting prospects for improving treatment options and outcomes in CNS-related disorders (Satapathy et al, 2021).
​
​
​
LNPs have shown to be a transformative technology for drug delivery and gene therapy as well as having other applications in diagnostics and imaging. The structure of LNPs makes them useful tools in medicine as they are easy to produce in large quantities, are biodegradable and have the potential to deliver drugs to targeted locations. They have also been explored for delivery of drugs across the blood brain barrier, showing promise for treatments of neurodegenerative diseases. As we gain a better understanding of lipid chemistry and structure-function relationships, the future design of LNPs for drug delivery is poised to become even more effective, offering hope for enhanced therapeutic outcomes in the years to come.
Delivery of Genetic Material
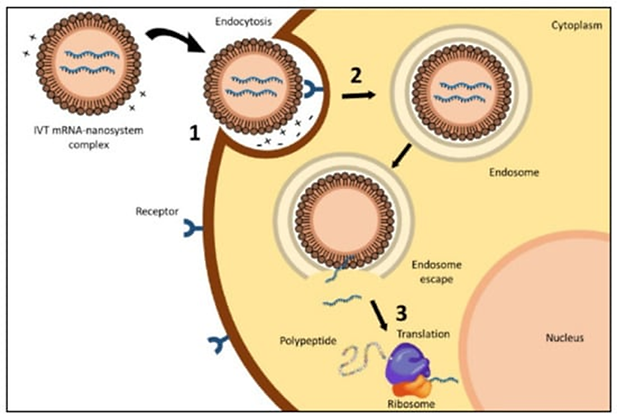
Delivery of Drug targets
Key Applications and Versatility
Conclusion
References
Aldosari, B. N., Alfagih, I. M., & Almurshedi, A. S. (2021). Lipid Nanoparticles as Delivery Systems for RNA-Based Vaccines. Pharmaceutics, 13(2), 206. MDPI AG. http://dx.doi.org/10.3390/pharmaceutics13020206
​
Akita, T., Oda, K., Narukawa, S., Morita, Y., Tange, K., Nakai, Y., & Yamashita, C. (2023). Intracellular Drug Delivery Process of Am80-Encapsulated Lipid Nanoparticles Aiming for Alveolar Regeneration. Pharmaceuticals, 16(6), 838. MDPI AG. Retrieved from http://dx.doi.org/10.3390/ph16060838
Allen, T. M., & Cullis, P. R. (2013). Liposomal drug delivery systems: from concept to clinical applications. Advanced drug delivery reviews, 65(1), 36–48. https://doi.org/10.1016/j.addr.2012.09.037
​
Bangham, A. D., Standish, M. M., & Watkins, J. C. (1965). Diffusion of univalent ions across the lamellae of swollen phospholipids. Journal of Molecular Biology,13(1), 238-252.
​
Bangham, A. D., Standish, M. M., Watkins, J.C. & Weissmann, G. (1967). The diffusion of ions from a phospholipid model membrane system, Protoplasma 63, 183–187.
​
Bao, Y., Jin, Y., Chivukula, P., Zhang, J., Liu, Y., Liu, J., Clamme, J. P., Mahato, R. I., Ng, D., Ying, W., Wang, Y., & Yu, L. (2013). Effect of PEGylation on biodistribution and gene silencing of siRNA/lipid nanoparticle complexes. Pharmaceutical research, 30(2), 342–351. https://doi.org/10.1007/s11095-012-0874-6
​
Brader, M. L., Williams, S. J., Banks, J. M., Hui, W. H., Zhou, Z. H., & Jin, L. (2021). Encapsulation state of messenger RNA inside lipid nanoparticles. Biophysical journal, 120(14), 2766–2770. https://doi.org/10.1016/j.bpj.2021.03.012
​
Cárdenas, M., Campbel, R. A., Arteta, M. Y., Lawrence, M. J., Sebastiani, F. (2023). Review of structural design guiding the development of lipid nanoparticles for nucleic acid delivery. Current Opinion in Colloid & Interface Science, 66. 101705. https://doi.org/10.1016/j.cocis.2023.101705
​
Cheng, X., Lee, R.J. (2016). The role of helper lipids in lipid nanoparticles (LNPs) designed for oligonucleotide delivery. Adv. Drug. Deliv. Rev, 99:129–137.
​
Cullis, P. R., & Hope, M. J. (2017). Lipid Nanoparticle Systems for Enabling Gene Therapies. Molecular therapy: the journal of the American Society of Gene Therapy, 25(7), 1467–1475. https://doi.org/10.1016/j.ymthe.2017.03.013
​
Dhiman, N., Awasthi, R., Sharma, B., Kharkwal, H., & Kulkarni, G. T. (2021). Lipid nanoparticles as carriers for bioactive delivery. Frontiers in Chemistry, 9. https://doi.org/10.3389/fchem.2021.580118
​
Eldem, T., Speiser, P., & Hıncal, A. (1991). Optimization of Spray-Dried and -Congealed Lipid Micropellets and Characterization of Their Surface Morphology by Scanning Electron Microscopy. Pharmaceutical Research, 08(1), 47–54. https://doi.org/10.1023/a:1015874121860
​
Gasco, M.R. (1997) Method for producing solid lipid nanospheres with warm microemulsions. Pharm Tech Eur 9: 52-58
​
Garud, A., Singh, D., & Garud, N. (2012). Solid lipid nanoparticles (SLN): Method, Characterization and applications. International Current Pharmaceutical Journal, 1(11), 384–393. https://doi.org/10.3329/icpj.v1i11.12065
​
Ghasemiyeh, P., & Mohammadi-Samani, S. (2018). Solid lipid nanoparticles and nanostructured lipid carriers as novel drug delivery systems: applications, advantages, and disadvantages. Research in Pharmaceutical Sciences, 13(4), 288. https://doi.org/10.4103/1735-5362.235156
​
Gregoradis, G. a (1976a), The carrier potential of liposomes in biology and medicine. Part 1, N. Engl. J. Med. 295, 704–710.
​
Gregoriadis, G. b (1976b). The carrier potential of liposomes in biology and medicine. Part 2, N. Engl. J. Med. 295, 765–770.
​
Gregoriadis, G, & Ryman, B.E. (1971). Liposomes as carriers of enzymes or drugs: a new approach to the treatment of storage diseases, Biochem. J. 124.
​
Hald Albertsen, C., Kulkarni, J. A., Witzigmann, D., Lind, M., Petersson, K., & Simonsen, J. B. (2022). The role of lipid components in lipid nanoparticles for vaccines and gene therapy. Advanced drug delivery reviews, 188, 114416. https://doi.org/10.1016/j.addr.2022.114416
​
Han, J., Lim, J., Wang, CP.J. et al. Lipid nanoparticle-based mRNA delivery systems for cancer immunotherapy. Nano Convergence 10, 36 (2023). https://doi.org/10.1186/s40580-023-00385-3
​
Hajj, K., & Whitehead, K. (2017). Tools for translation: non-viral materials for therapeutic mRNA delivery. Nature Reviews Materials 2, 17056. https://doi.org/10.1038/natrevmats.2017.56
​
Holland, J.W., Hui, C., Cullis, P.R., Madden, T.D. (1996). Poly(ethylene glycol)–lipid conjugates regulate the calcium-induced fusion of liposomes composed of phosphatidylethanolamine and phosphatidylserine. Biochemistry, 35:2618–2624.
​
Jaiswal, P., Gidwani, B., & Vyas, A. (2016). Nanostructured lipid carriers and their current application in targeted drug delivery. Artificial cells, nanomedicine, and biotechnology, 44(1), 27–40. https://doi.org/10.3109/21691401.2014.909822
​
Jung, H. N., Lee, S. Y., Lee, S., Youn, H., & Im, H. J. (2022). Lipid nanoparticles for delivery of RNA therapeutics: Current status and the role of in vivo imaging. Theranostics, 12(17), 7509–7531. https://doi.org/10.7150/thno.77259
Kim, H., & Leal, C. (2015). Cuboplexes: Topologically Active siRNA Delivery. ACS Nano 9, 10, 10214–10226. https://doi.org/10.1021/acsnano.5b03902
​
Khan, S., Sharma, A., & Jain, V. (2023). An Overview of Nanostructured Lipid Carriers and its Application in Drug Delivery through Different Routes. Advanced pharmaceutical bulletin, 13(3), 446–460. https://doi.org/10.34172/apb.2023.056
​
Kraft, J. C., Freeling, J. P., Wang, Z., & Ho, R. J. (2014). Emerging research and clinical development trends of liposome and lipid nanoparticle drug delivery systems. Journal of pharmaceutical sciences, 103(1), 29–52. https://doi.org/10.1002/jps.23773
​
Kularatne, R. N., Crist, R. M., & Stern, S. T. (2022). The Future of Tissue-Targeted Lipid Nanoparticle-Mediated Nucleic Acid Delivery. Pharmaceuticals, 15(7), 897. MDPI AG. http://dx.doi.org/10.3390/ph15070897
​
Leong, E. W. X., & Ge, R. (2022). Lipid Nanoparticles as Delivery Vehicles for Inhaled Therapeutics. Biomedicines, 10(9), 2179. https://doi.org/10.3390/biomedicines10092179
​
Let’s talk about lipid nanoparticles (2021). Nature Reviews Material 6, 99. https://doi.org/10.1038/s41578-021-00281-4
​
Lucks, J.S., & Müller, R.H., (1991). Medication vehicles made of solid lipid particles (solid lipid Nanospheres SLN). EP0000605497.
​
Mehnert, W., & Mäder, K. (2001). Solid lipid nanoparticles Production, characterization and applications. Advanced Drug Delivery Reviews, 47(2–3), 165–196. https://doi.org/10.1016/s0169-409x(01)00105-3
​
Mohammadi-Samani, S., & Ghasemiyeh, P. (2018). Solid lipid nanoparticles and nanostructured lipid carriers as novel drug delivery systems: applications, advantages and disadvantages. Research in Pharmaceutical Sciences, 13(4), 288. https://doi.org/10.4103/1735-5362.235156
​
Mukai, H., Ogawa, K., Kato, N., & Kawakami, S. (2022). Recent advances in lipid nanoparticles for delivery of nucleic acid, mRNA, and gene editing-based therapeutics. Drug metabolism and pharmacokinetics, 44, 100450. https://doi.org/10.1016/j.dmpk.2022.100450
​
Müller, R. H., Mäder, K., & Gohla, S. (2000). Solid lipid nanoparticles (SLN) for controlled drug delivery - a review of the state of the art. European journal of pharmaceutics and biopharmaceutics : official journal of Arbeitsgemeinschaft fur Pharmazeutische Verfahrenstechnik e.V, 50(1), 161–177. https://doi.org/10.1016/s0939-6411(00)00087-4
​
Müller, R. H., Radtke, M., & Wissing, S. A. a (2002a). Nanostructured lipid matrices for improved microencapsulation of drugs. International Journal of Pharmaceutics, 242(1-2), 121-128.
​
Müller, R.H., Radtke, M., & Wissing, S. A. b (2002b). Solid lipid nanoparticles (SLN) and nanostructured lipid carriers (NLC) in cosmetic and dermatological preparations. Advanced Drug Delivery Reviews, 54 (Supplement 1), S131-S155.
​
Naseri, N., Valizadeh, H., & Zakeri-Milani, P. (2015). Solid Lipid Nanoparticles and Nanostructured Lipid Carriers: Structure, Preparation, and Application. Advanced pharmaceutical bulletin, 5(3), 305–313. https://doi.org/10.15171/apb.2015.043
​
Oliveira, C., Ferreira, C. J. O., Sousa, M., Paris, J. L., Gaspar, R., Silva, B. F. B., Teixeira, J. A., Ferreira-Santos, P., & Botelho, C. M. (2022). A Versatile Nanocarrier-Cubosomes, Characterization, and Applications. Nanomaterials (Basel, Switzerland), 12(13), 2224. https://doi.org/10.3390/nano12132224
​
Pardeike, J., Hommoss, A., & Müller, R. H. (2009). Lipid nanoparticles (SLN, NLC) in cosmetic and pharmaceutical dermal products. International journal of pharmaceutics, 366(1-2), 170–184. https://doi.org/10.1016/j.ijpharm.2008.10.003
​
Pardi, N., Hogan, M. J., Porter, F. W., & Weissman, D. (2018). mRNA vaccines — a new era in vaccinology. Nature Reviews Drug Discovery, 17(4), 261–279. https://doi.org/10.1038/nrd.2017.243
​
Puri, A., Loomis, K., Smith, B., Lee, J. H., Yavlovich, A., Heldman, E., & Blumenthal, R. (2009). Lipid-based nanoparticles as pharmaceutical drug carriers: from concepts to clinic. Critical reviews in therapeutic drug carrier systems, 26(6), 523–580. https://doi.org/10.1615/critrevtherdrugcarriersyst.v26.i6.10
​
Satapathy, M. K., Yen, T. L., Jan, J. S., Tang, R. D., Wang, J. Y., Taliyan, R., & Yang, C. H. (2021). Solid Lipid Nanoparticles (SLNs): An Advanced Drug Delivery System Targeting Brain through BBB. Pharmaceutics, 13(8), 1183. https://doi.org/10.3390/pharmaceutics13081183
​
Schoen, A. H. (1970). Infinite periodic minimal surfaces without self-intersections (PDF) (Technical report). NASA Technical Note. NASA TN D-5541.
​
Schwarz, C., Mehnert, W., Lucks, J., & Müller, R. H. (1994). Solid lipid nanoparticles (SLN) for controlled drug delivery. I. Production, characterization and sterilization. Journal of Controlled Release, 30(1), 83–96. https://doi.org/10.1016/0168-3659(94)90047-7
Scioli Montoto, S., Muraca, G., & Ruiz, M. E. (2020) Solid Lipid Nanoparticles for Drug Delivery: Pharmacological and Biopharmaceutical Aspects. Front. Mol. Biosci. 7:587997. doi: 10.3389/fmolb.2020.587997
Semple, S. C., Akinc, A., Chen, J., Sandhu, A. P., Mui, B. L., Cho, C. K., Sah, D. W., Stebbing, D., Crosley, E. J., Yaworski, E., Hafez, I. M., Dorkin, J. R., Qin, J., Lam, K., Rajeev, K. G., Wong, K. F., Jeffs, L. B., Nechev, L., Eisenhardt, M. L., Jayaraman, M., … Hope, M. J. (2010). Rational design of cationic lipids for siRNA delivery. Nature biotechnology, 28(2), 172–176. https://doi.org/10.1038/nbt.1602
Sjöström, B., & Bergenståhl, B. (1992). Preparation of submicron drug particles in lecithin-stabilized o/w emulsions I. Model studies of the precipitation of cholesteryl acetate. International Journal of Pharmaceutics, 88(1–3), 53–62. https://doi.org/10.1016/0378-5173(92)90303-j
Sun, D., & Lu, Z. R. (2023). Structure and Function of Cationic and Ionizable Lipids for Nucleic Acid Delivery. Pharmaceutical research, 40(1), 27–46. https://doi.org/10.1007/s11095-022-03460-2
​
Tenchov, R., Bird, R. E., Curtze, A., & Zhou, Q. (2021). Lipid Nanoparticles─From liposomes to mRNA Vaccine delivery, a landscape of research diversity and advancement. ACS Nano, 15(11), 16982–17015. https://doi.org/10.1021/acsnano.1c04996
​
Torchilin V. P. (2010). Passive and active drug targeting: drug delivery to tumors as an example. Handbook of experimental pharmacology, (197), 3–53. https://doi.org/10.1007/978-3-642-00477-3_1
​
Torchilin, V. P. (2014). Multifunctional, stimuli-sensitive nanoparticulate systems for drug delivery. Nature Reviews Drug Discovery, 13(11), 813–827. https://doi.org/10.1038/nrd4333
​
Wang, X., Wu, D., & Senyo, S. E. (2022). mRNA therapy for myocardial infarction: A review of targets and delivery vehicles. Frontiers in Bioengineering and Biotechnology, 10. https://doi.org/10.3389/fbioe.2022.1037051
​
Xu, L., Wang, X., Liu, Y., Yang, G., Falconer, R.J. and Zhao, C. (2022). Lipid Nanoparticles for Drug Delivery. Advanced NanoBiomed Research. 2: 2100109. https://doi.org/10.1002/anbr.202100109
​
Yin, H., Kanasty, R., Eltoukhy, A., Vegas, A. J., Dorkin, J. R., Anderson, D. G. (2014). Non-viral vectors for gene-based therapy. Nature Reviews Genetics 15, 541–555. https://doi.org/10.1038/nrg3763
​
Yizhak, K., Aguet, F., Kim, J., Hess, J. M., Kübler, K., Grimsby, J., Frazer, R., Zhang, H., Haradhvala, N. J., Rosebrock, D., Livitz, D., Li, X., Arich-Landkof, E., Shoresh, N., Stewart, C., Segrè, A. V., Branton, P. A., Polak, P., Ardlie, K. G., & Getz, G. (2019). RNA sequence analysis reveals macroscopic somatic clonal expansion across normal tissues. Science, 364(6444), eaaw0726. https://doi.org/10.1126/science.aaw0726
​
Zhan, S. M., Hou, D. Z., Ping, Q. N., & Xu, Y. (2010). Preparation and entrapment efficiency determination of solid lipid nanoparticles loaded levodopa. Chin. J. Hosp. Pharm, 14, 1171-1175.
​
Zuhorn, I., Hoekstra, D. (2002). On the Mechanism of Cationic Amphiphile-mediated Transfection. To Fuse or not to Fuse: Is that the Question? . J. Membrane Biol. 189, 167–179. https://doi.org/10.1007/s00232-002-1015-7